Technical challenges of linac-based stereotactic ablative body radiotherapy: short review for non-radiation oncologists
Introduction
Stereotactic ablative body radiotherapy (SABR) or stereotactic body radiotherapy (SBRT) is a radiation technique that delivers very high doses of radiation (7.5–24 Gy per fraction), in a small number of treatments (1-8), to extra-cranial targets. SABR is currently the standard of care for patients with inoperable early stage non-small cell lung cancer (i.e., inoperable because of medical comorbidities) or for those who opt against surgery (1,2). In these patients, SABR results in long-term survival outcomes that are similar to surgery, with low risk of toxicity and little detriment to quality of life (3-6). SABR is also increasingly being used in patients with oligometastatic, oligo-recurrent or oligoprogressive malignancies.
SABR is also increasingly being used in patients with oligometastatic, oligo-recurrent or oligoprogressive malignancies. The concept of oligometastatic disease was initially defined in 1995 by Hellman and Weichselbaum (7). It represents an intermediate state between the localized disease and wide spread metastatic disease, with more indolent course. Resection or ablative treatments of all sites of known limited metastatic disease could result in prolonged disease free survival with the potential for cure in these patients (8). The most commonly accepted definition of oligometastatic disease refers to less than 5 metastases in less than 3 organs. While a consensus definition of the oligometastatic state has not been reached, some have further subdivided patients based on the temporal development or progression of metastases in regards to diagnosis and systemic therapy (9). De novo (also called synchronous) oligometastases refer to the presence of few metastases at time of initial diagnosis, oligorecurrence (also called metachronous oligometastases) refers to the development of few metastases after definitively treating the primary site, while induced or persistent oligometastases describes residual metastatic lesions after response to systemic therapy. Oligoprogression describes a patient with widespread metastases with relatively stable disease on systemic therapy with only one or a few lesions demonstrating growth.
Randomized phase 2 trials (10-12) have demonstrated that SABR to all apparent sites of oligometastases is associated with significant improvement of progression free survival and overall survival compared to standard of care therapy. Notably, SABR to sites of oligoprogression delays the time to further progression, and allows for continuation of systemic therapy that has been mostly effective (in all but the sites of oligoprogression). SABR is also used for recurrent head and neck cancers (13), as a curative treatment option for prostate cancer (14-16) and unresectable kidney cancers (17,18), and is being investigated as an adjuvant to surgery for early stage breast cancer (19,20) and other indications. Table 1 presents the main indications of SABR in current clinical practice
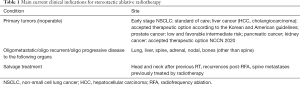
Full table
The term stereotaxy refers to the ability to link the patient geometry to an external coordination system, for precise target localization of the disease. With modern SABR techniques, internal coordinates (implanted fiducials or 3-dimensional imaging) have been used. Ablation (in the context of radiotherapy) implies the delivery of very high doses of radiation that are able to induce cell kill by a variety of mechanisms. The high fractional doses of radiation may have some biologic benefits such as the ability to overcome intratumoral regional hypoxia as well as potentially stimulating an immune response (21).
The differences between conventional radiotherapy and SABR are presented in Table 2.

Full table
The main advantage of SABR is a steep dose gradient, that is, a rapid dose fall-off outside the target, allowing delivery of high biologically effective doses to the target, while minimizing irradiation exposure of the neighboring normal tissues. This results in high rates of local control of the treated target and minimal toxicity risks. SABR is a non-invasive or minimally invasive technique, which does not require a hospital stay, and it is associated with low morbidity and little impact on the quality of life of patients. It is suitable for patients who are poor candidates for surgery or other ablative techniques.
However, the steep dose gradient achieved with SABR also represents a technical challenge, as set-up errors, intra-fraction motion and respiratory motion of the target can lead to under-dosing of the target and/or unwanted irradiation of normal tissues, and therefore increase the risk of toxicity, particularly with the relatively high biologically effective doses that are used. This can be especially critical for targets situated near the spinal cord, duodenum/stomach or central thoracic structures. Therefore, all efforts should be made to reduce these uncertainties. Currently available practice guidelines from the American College of Radiology (ACR) and American Society of Radiation Oncology (ASTRO) (22), and American Association of Physicists in Medicine (AAPM) (23), emphasize that the overall process of SABR requires strict protocols in treatment simulation, planning and delivery.
In addition, while modern technologies allow for SABR to be delivered in a course of days as opposed to weeks (with conventional radiotherapy), the duration of the individual treatments (with additional effort required for precise immobilization, and monitoring of the patient and/or target before and during treatment) remains long (15–60 minutes) compared to the conventional radiation techniques (generally on the order of 5–15 minutes). Therefore, comfort and compliance of patients undergoing SABR are of paramount importance. Table 3 presents a synopsis of the main requirements for SABR.
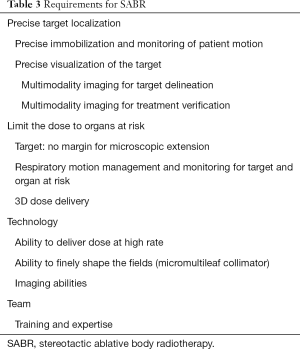
Full table
General principles of SABR treatment planning and delivery will be presented in the next sections, with emphasis on the strategies to reduce errors related to immobilization, respiratory management and treatment verification.
Immobilization
Comfortable immobilization of the patient reduces the risk of inter and intra-fraction errors. Several immobilization devices available are commercially available, with reported repositioning accuracy between 0.3–5.6 mm (23). Body frames (Figure 1A) usually use vacuum cushions to physically immobilize patients, while maintaining comfort. Additionally, some groups use abdominal compression devices (Figure 1B) to reduce the motion of the targets/organs at risk in areas with known large motion (lower lungs, upper abdomen); however, abdominal compression is often uncomfortable and has been shown to deform anatomy, depending on the positioning and the pressure applied. Furthermore, abdominal compression cannot be used for patients with abdominal co-morbidities, such as aortic aneurysms or gastrostomies. Some groups from Scandinavia advocate no immobilization, favoring patient comfort. However, while current imaging systems allow for detection and correction in patient positioning, they cannot replace proper immobilization of the patients. Accurate immobilization is critical in patient undergoing spine SBRT: studies assessing different immobilization devices, suggest that the use of semirigid vacuum immobilization (body frame) is associated with the least intra-fraction errors (compared to evacuated cushion or thermoplastic S-frames) (24).
Generally, patients are set-up in a dorsal decubitus position, with their arms above the head (unless treating a target in the head and neck region (Figure 1B) or lower abdomen or pelvis). Removing the arms from the beam entry is generally necessary, since imprecise arm positioning will affect the accuracy of dosimetry; therefore, elevating the arms allows for more flexibility in the choice of beam directions. However, this position can be associated with positional pain in the shoulders and upper back, especially in the context of long treatment times. Such discomfort can cause patient motion during treatment, and therefore increase the risk of errors in dose delivered.
Simulation
Simulation is an essential part of the radiotherapy process. For SABR, most centers will use CT scans for simulation, with few using MRIs or PET-CT at the time of simulation. Diagnostic imaging (including PET-CT and MRI) obtained prior to or after simulation can be registered to the images obtained at simulation (25). If feasible, attempts to position the patient for diagnostic imaging similarly (i.e., using a flat board) to the position in simulation will facilitate better image registration. However, there are deformable image fusion protocols that allow reasonably good fusion (to selected regions) even with different positioning. For SABR, the recommended CT slice thickness for simulation is ~1.25 mm at the level of the targets, and 2.5 mm remaining of the scan, though individual practices vary.
Respiratory correlated CT scan (4-dimensional CT or 4DCT) is the recommended CT for SABR planning. Respiratory correlated scans are acquired during several respiratory cycles, in a manner that is described as ‘over-sampled.’ Individual CT images are placed into discrete groups (generally 1 of 10) that correspond to a position within the respiratory cycle. This process will allow determination of the respiratory motion of the target and normal tissues; often a reconstructed video of the motion can be displayed showing this movement. If 4DCT is not available, a slow acquisition CT, CT at extremes of respiration, or even fluoroscopy can be used to determine the extent of the respiratory motion of the target. In patients with tumors in the lower lung lobes or upper abdomen, as well as in case of large tumor motion, breathhold CT scans can be recommended if patient is compliant and able to hold his/her breath for at least 20 seconds (more details below). The advantages and disadvantages of each imaging modality are detailed in the Table 4.
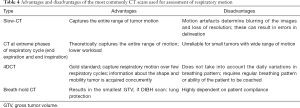
Full table
Tumors in the liver, especially metastatic, can be difficult to differentiate from the normal liver parenchyma. Therefore, small fiducial markers can be inserted near the tumor, for easier identification of the target on the CT simulation and CBCT. However, insertion of fiducial markers is an invasive procedure, requires day admission and rarely can be associated with complications (e.g., hemorrhage, hematoma, misplacement, migration, infection, etc.) (26-28).
Respiratory motion and its management
Respiratory motion is an important source of uncertainty in radiotherapy. It is patient-specific. Individual respiratory characteristics can vary in amplitude, period and regularity during and between SABR fractions (intra-fraction, inter-fraction). In addition, it was shown that over the course of treatment, tumors may change in size, shape and mobility. AAPM TG 76 (29) and 101 (23) recommend assessment of respiratory motion in all patients treated with SABR, while the respiratory management should be individualized. Respiratory management is recommended for all tumors moving more than 5mm in any direction. Dosimetric studies have shown that for tumor motion >5 mm, the interplay effect can result in underdosing of the PTV when IMRT techniques are used (30). This interplay effect is less significant when 3D CRT technique is used (same “intensity” of the beam). In our practice, respiratory management is recommended for all tumors moving more than 5 mm in any direction, when IMRT or rotational techniques are used.
Several types of management of respiratory motion have been described in the literature. They can be grouped in two main categories:
- Passive management (motion encompassing techniques): takes into account the total range of motion and requires knowledge of the motion extent. 4DCT (discussed above) is typically used to determine the magnitude of motion. If one were to treat a target that encompassed every position within the 4DCT scan, often the target is quite large (especially for patients with tumors in the lower lobes of the lung, liver, etc.), and can result in unwanted radiation of the neighboring normal structures.
- Active management: involves either limitation of the respiratory motion (breathhold techniques, abdominal compression) or monitoring the target or patient during treatment and adjusting the treatment. This can be accomplished with respiratory gating (discussed more below), in which the treatment is delivered only when the patient’s breathing is within certain phases of the respiratory cycle (usually within a relaxed breathing window) or tracking, in which the treatment machine adjusts the treatment based on the patient position. Patients can be monitored during treatment with either an external or an internal marker of motion, or the imaging of the targeted tumor. Different correlations between external markers and internal tumor motion are reported in the literature (31-33). While active management usually results in smaller target volumes, and therefore lesser irradiation of neighboring normal structures, it is also associated with increased treatment time, therefore comfort and optimization of the patient before the treatment is mandatory. For example, patients with arthritis might require antalgic before treatment in order to be able to maintain the required treatment position; some patients might require oxygen during the treatment, etc.
Determination of required respiratory management
- For targets moving less than 5 mm in any direction-free breathing non-gated treatment can be considered. This is the most widely used technique and the easiest to implement.
- For targets with motion over 5 mm in any direction the following can be implemented:
- Respiratory gating, which requires a regular respiratory pattern, minimizes the effect of respiratory motion, by limiting the delivery of the treatment beam during specific portions (“gate”) of the respiratory cycle. Gating can reduce the volume of the neighboring normal tissue receiving unnecessary irradiation. Notably, it increases treatment time by a factor of ~3. If the respiratory gate is too narrow (i.e., treatment only within a narrow range of the respiratory cycle), then it becomes impractical, and another method should be used.
- Breathhold is a variation of gated treatment, with the treatment being delivered only when the patient is holding their breath either in deep inspiration, deep expiration, or relaxed ‘end expiration’; the latter is reported (34,35) as the most reproducible, though the choice of breath-hold technique depends on patient compliance as well as how the normal tissues move relative to the target (e.g., one approach may better move the heart or liver away from a target). It is highly dependent on patient compliance and ability (i.e., their baseline respiratory function). Breathhold allows significant reduction of the set-up uncertainty, which reduces unnecessary irradiation of normal tissues (36). However, patients must be able to hold their breath for at least 15–20 seconds. It requires imaging-based treatment verification (i.e., fluoroscopy during breathhold, or surface imaging) to assure that the patient does not move or breath during treatment.
- If a patient is unable to comply with breath-hold, and exhibits an irregular respiratory cycle pattern, monophasic (patient instructed when to inhale and exhales at own pace) or biphasic (patient instructed when to inhale and exhale) coaching of the patient might be required. This is highly dependent on the ability of the patient to follow commands, and patient compliance. Coaching allows regular breathing, therefore possibility to gate, however the amplitude of motion increases, and quality of the acquired imaging is decreased (more motion artefacts).
- For patients with irregular breathing, unable to breathhold or follow commands, a 3D-SBRT free breathing approach should be employed.
In addition, for tumors situated near sensitive critical structures, the motion of the nearby organs at risk should be analyzed and considered. For tumors in the upper abdomen, in addition to the respiratory motion, other factors to be considered are stomach fullness and peristalsis. For tumors situated in the proximity of the stomach, treatment with empty stomach is recommended.
Treatment planning
For patients undergoing radiotherapy, definition of the target and normal tissues [also called organs at risk (OAR)] is an essential part of the entire treatment process. Guidelines for the definition of the target volumes and OARs are currently available (23,29). The gross tumor volume (GTV) is defined by the Radiation Oncologist using anatomical and/or metabolic imaging (CT, PET, MRI). For SABR, it is customary not to define a clinical target volume (CTV), which considers the microscopic extension of the tumor. The internal target volume (ITV) takes into account the respiratory motion of the target, while the planning target volume (PTV) is defined to account for setup error. Figure 2 presents an example of target definition for SABR. The OAR is often defined according to available contouring atlases (37).
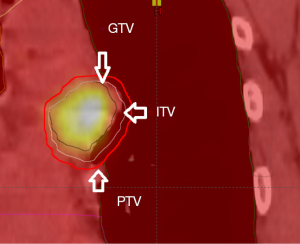
In order to maximize the dose received by the target and minimize the exposure of the OARs the delivered dose must be geometrically spread, by using multiple radiation beams, as well as conformal radiation techniques: 3D-conformal RT, fixed gantry intensity modulated radiotherapy (IMRT) or rotational techniques [tomotherapy, volumetric modulated arc therapy (VMAT)] (Figure 3).
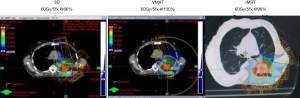
There is significant variability, depending on diagnosis and individual institutional policies, in the prescribed dose and fractionation, as well as in the acceptable OAR dosimetric constraints used (18,38-46). The choice of prescribed dose fractionation is driven mainly by the position of the tumor relative to critical organs nearby, with steep dose gradients required. Documentation of the dose received, normal tissue dose exposure, and technique utilized (including type of respiratory management) are essential, especially if re-irradiation will potentially be necessary, which is a common scenario for patients with cancer, particularly those with oligometastatic disease (47,48).
Several review articles have summarized published literature in an attempt to better define normal tissue complication probabilities (NTCP) and acceptable normal tissue dose constraints after SBRT (21,23,40,49,50). This remains a complicated area of study. The AAPM TG 101 report (23) provides recommended dose-volume constraints for normal tissues, many of which are incorporated into ongoing cooperative group randomized trials. We recommend radiation oncologists and physicists adhere to the published guidelines from AAPM TG101 and the UK Consortium (51). Future research efforts should be directed towards optimizing treatment parameters, such as fractional dose and total dose, as well as specific normal tissue dose-volume constraints.
The plan is reviewed by the Radiation Oncologist, together with the Physicists and/or Dosimetrist to ensure that all requirements for target and OARs are met. In patients with multiple targets treated concurrently, care should be taken when assessing the treatment plan: dose conformity should be assessed for the individual targets, however the OAR should be evaluated on the composite plan (plan sum), to assess the contribution of each plan to each OAR.
Following acceptance of the plan by the Radiation Oncologist, a rigorous quality assurance process is performed to verify the accuracy of the treatment plan delivery. This patient-specific verification of the treatment plan can be performed using several different evaluation techniques and will depend on which treatment technique has been selected 3D-CRT, IMRT or VMAT. The AAPM TG 218 report (52) defines the methodology and tolerances required for a comprehensive and consistent pre-treatment verification programme.
Treatment verification
Verification of the patient positioning and target location before the treatment beam is turned on is an essential part of any radiotherapy treatment. Traditionally, MV or kV 2-dimensional images were used to verify the conventional treatments. However, with the complex treatments such as SABR, image guidance involves more sophisticated imaging modalities, especially when uncertainties related with respiratory motion are taken into account (53,54). These include:
- X-ray imaging using MV or kV photons.
- 3D and 4D imaging (cone beam CT-CBCT).
- Newer technologies including imaging and monitoring of electromagnetic transponders (55-57) or small fiducial markers inserted near the tumor and visible on X-rays or CBCT (58-62).
- Lately surface guidance is used for verification and monitoring of the patient set-up (63,64).
The next paragraphs will focus on the most widely used methods of treatment verification.
While kV imaging can be used for rapid verification of the isocenter, or alignment of bony anatomy (if obtained orthogonally—meaning 90 degree separation) the verification of the target and OARs positioning often requires volumetric images (65-68) (such as cone beam CT or CBCT). CBCT uses an imaging source and array of detectors (incorporated onto the linear accelerator) that rotate (generally 180 degrees) around a patient to generate planar imaging; CBCT is the most readily available imaging modality for online image guidance. Standard CBCTs are 3D imaging modalities, often acquired over few respiratory cycles. Therefore, caution should be used in interpretation of the data, especially when 4DCT was used to define the target. The target image might be blurred, as the CBCT will “record” the target position over few respiratory cycles (69-70). Figure 4 presents an example of the use of CBCT for treatment verification.
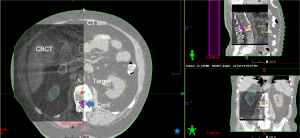
Some newer linear accelerators are equipped with respiratory correlated CBCT (4DCBCT) which could be then compared with the 4DCT simulation image set. The image acquisition time can be longer (71), which can be an issue especially for unfit patients. However, 4DCBCT are especially useful for small tumors with large motion, or for tumors situated near the diaphragm (70,72,73).
The online image verification is used to insure that the target is covered within the predefined PTV, and that the organs at risk are outside predefined critical doses for a specific outcome (see Figure 5).
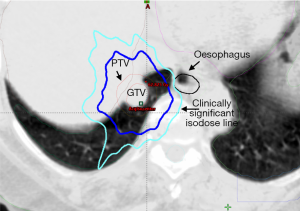
Another online imaging modality that can be used is fluoroscopy. This imaging modality should be used when gating is used for respiratory management, to verify that the tumor stays within the predefined gate. It is especially important for small tumors with significant motion, which can be underestimated by CBCT (74,75). The drawback of fluoroscopy is that it is a 2D imaging modality, however, used in conjunction with CBCT it is a very quick and elegant modality to verify gated treatments. In addition, it is a useful tool that can identify if there are any phase -delays between the surrogate and the tumor, which cannot be otherwise seen.
Regardless of the imaging modality used, the verification has to be repeated if the patient moves or if there are significant changes of the respiratory pattern.
A multidisciplinary team composed of Radiation Oncologist, Physicist and Radiation Therapists should be present at the time of treatment verification. Once the team is satisfied that all parameters of the treatment correspond to what was planned, the treatment can be delivered. If the treatment delivery is long, one might consider re-imaging mid-treatment and at the end of the session, to insure that there was not intra-fraction motion of the target.
While the methods for treatment verification are improving and become more sophisticated, one should not forget that positioning verification requires additional time for the patient in treatment positions that can be uncomfortable. The verification time should be minimized to decrease the likelihood of errors induced by patient motion.
Novel technologies for SBRT
This article focused mainly on the technical aspects of the most widely available SABR technique, using modern linear accelerators (LINACs). In the early 2000s, Cyberknife® was introduced. Cyberknife is a delivery system which allows for SABR, using real time monitoring of fiducials of bony anatomy, coupled with robotically controlled tracking of the target/patient. The more recent development of the MRI-based treatment delivery systems (with cobalt 60 radioactive sources or LINAC) similarly allows for improved accuracy of patient set-up and monitoring of intra-fraction motion; the incorporation of MR imaging also allows for adaptation of the treatment to inter-fraction anatomy changes and real time adaptation of the treatment delivery. Several teams published data for lung, liver, pancreatic, spinal tumors (76-78). However, this technique is unfortunately not widely available.
Furthermore, recently a new concept is developing namely biology guided radiotherapy. In 2018 (79), at the ASTRO meeting, a PET-integrated linac was presented Use of a small amount of a radioactive tracer (most commonly FDG), signals the tumor location and highlights the differences between healthy cells and cancer cells. By allowing real-time identification of these emissions, this new LINAC would allow real time tracking of the tumor and therefore a new way to manage the respiratory motion without additional margins. Theoretically, this will result in minimisation of the exposure of the normal tissues to radiation, improved toxicity profile, better quality of life of these patients. This new machine is not yet in use in clinical practice.
Conclusions
SABR is a highly efficacious treatment, associated with high local control, minimal toxicity and minimal impact on the quality of life of the patients. However, SABR requires advanced technology and accuracy at all steps involved, from simulation to planning and treatment delivery. Achieving high accuracy can be challenging especially in patients with significant comorbidities, especially if long treatment times are required. All efforts should be made to minimize the treatment times, and improve the comfort of the patients.
Acknowledgments
Funding: None.
Footnote
Provenance and Peer Review: This article was commissioned by the editorial office, Annals of Palliative Medicine for the series “Oligometastasis- Fallacy or Real Deal?”. The article has undergone external peer review.
Peer Review File: Available at http://dx.doi.org/10.21037/apm-20-950
Conflicts of Interest: All authors have completed the ICMJE uniform disclosure form (available at http://dx.doi.org/10.21037/apm-20-950). The series “Oligometastasis- Fallacy or Real Deal?” was commissioned by the editorial office without any funding or sponsorship. MTM served as the unpaid Guest Editor of the series. MTM reports royalties from Wolters Kluwer and honorarium from Galera Therapeutics, outside the submitted work. The authors have no other conflicts of interest to declare.
Ethical Statement: The authors are accountable for all aspects of the work in ensuring that questions related to the accuracy or integrity of any part of the work are appropriately investigated and resolved.
Open Access Statement: This is an Open Access article distributed in accordance with the Creative Commons Attribution-NonCommercial-NoDerivs 4.0 International License (CC BY-NC-ND 4.0), which permits the non-commercial replication and distribution of the article with the strict proviso that no changes or edits are made and the original work is properly cited (including links to both the formal publication through the relevant DOI and the license). See: https://creativecommons.org/licenses/by-nc-nd/4.0/.
References
- NCCN Clinical Practice Guidelines in Oncology-Non small cell lung cancer [Internet] 2020. Available online: https://www.nccn.org/professionals/physician_gls/pdf/nscl.pdf
- Haasbeek CJA, Palma D, Visser O, et al. Early-stage lung cancer in elderly patients: a population-based study of changes in treatment patterns and survival in the Netherlands. Ann Oncol 2012;23:2743-7. [Crossref] [PubMed]
- Lagerwaard FJ, Verstegen NE, Haasbeek CJA, et al. Outcomes of stereotactic ablative radiotherapy in patients with potentially operable stage I non-small cell lung cancer. Int J Radiat Oncol Biol Phys 2012;83:348-53. [Crossref] [PubMed]
- Crabtree TD, Puri V, Robinson C, et al. Analysis of first recurrence and survival in patients with stage I non-small cell lung cancer treated with surgical resection or stereotactic radiation therapy. J Thorac Cardiovasc Surg 2014;147:1183-91; discussion 1191-2. [Crossref] [PubMed]
- Shirvani SM, Jiang J, Chang JY, et al. Lobectomy, Sublobar Resection, and Stereotactic Radiation for Early-Stage Non-Small Cell Lung Cancers in the Elderly. JAMA Surg 2014;149:1244-53. [Crossref] [PubMed]
- Bahig H, Chen H, Louie AV. Surgery versus SABR for early stage non-small cell lung cancer: the moving target of equipoise. J Thorac Dis 2017;9:953-6. [Crossref] [PubMed]
- Hellman S, Weichselbaum RR. Oligometastases. J Clin Oncol 1995;13:8-10. [Crossref] [PubMed]
- Hellman S. Evolving paradigms and perceptions of cancer. Nat Clin Pract Oncol 2005;2:618-24. [Crossref] [PubMed]
- Patel PR, Yoo DS, Niibe Y, et al. A call for the aggressive treatment of oligometastatic and oligo-recurrent non-small cell lung cancer. Pulm Med 2012;2012:480961 [Crossref] [PubMed]
- Palma DA, Olson R, Harrow S, et al. Stereotactic ablative radiotherapy versus standard of care palliative treatment in patients with oligometastatic cancers (SABR-COMET): a randomised, phase 2, open-label trial. Lancet 2019;393:2051-8. [Crossref] [PubMed]
- Gomez DR, Blumenschein GR, Lee JJ, et al. Local consolidative therapy versus maintenance therapy or observation for patients with oligometastatic non-small-cell lung cancer without progression after first-line systemic therapy: a multicentre, randomised, controlled, phase 2 study. Lancet Oncol 2016;17:1672-82. [Crossref] [PubMed]
- Iyengar P, Wardak Z, Gerber DE, et al. Consolidative Radiotherapy for Limited Metastatic Non-Small-Cell Lung Cancer: A Phase 2 Randomized Clinical Trial. JAMA Oncol 2018;4:e173501 [Crossref] [PubMed]
- Vargo JA, Moiseenko V, Grimm J, et al. Head and Neck Tumor Control Probability: Radiation Dose-Volume Effects in Stereotactic Body Radiation Therapy for Locally Recurrent Previously-Irradiated Head and Neck Cancer: Report of the AAPM Working Group. Int J Radiat Oncol Biol Phys 2018;S0360-3016(18)30107-X.
- Cushman TR, Verma V, Khairnar R, et al. Stereotactic body radiation therapy for prostate cancer: systematic review and meta-analysis of prospective trials. Oncotarget 2019;10:5660-8. [Crossref] [PubMed]
- Ost P, Reynders D, Decaestecker K, et al. Surveillance or Metastasis-Directed Therapy for Oligometastatic Prostate Cancer Recurrence: A Prospective, Randomized, Multicenter Phase II Trial. J Clin Oncol 2018;36:446-53. [Crossref] [PubMed]
- Siva S, Bressel M, Murphy DG, et al. Stereotactic Abative Body Radiotherapy (SABR) for Oligometastatic Prostate Cancer: A Prospective Clinical Trial. Eur Urol 2018;74:455-62. [Crossref] [PubMed]
- Correa RJM, Louie AV, Zaorsky NG, et al. The Emerging Role of Stereotactic Ablative Radiotherapy for Primary Renal Cell Carcinoma: A Systematic Review and Meta-Analysis. Eur Urol Focus 2019;5:958-69. [Crossref] [PubMed]
- Siva S, Louie AV, Warner A, et al. Pooled analysis of stereotactic ablative radiotherapy for primary renal cell carcinoma: A report from the International Radiosurgery Oncology Consortium for Kidney (IROCK). Cancer 2018;124:934-42. [Crossref] [PubMed]
- Becker SJ, Niu Y, Mutaf Y, et al. Development and validation of a comprehensive patient-specific quality assurance program for a novel stereotactic radiation delivery system for breast lesions. J Appl Clin Med Phys 2019;20:138-48. [Crossref] [PubMed]
- Lozza L, Fariselli L, Sandri M, et al. Partial breast irradiation with CyberKnife after breast conserving surgery: a pilot study in early breast cancer. Radiat Oncol 2018;13:49. [Crossref] [PubMed]
- Milano MT, Constine LS, Okunieff P. Normal tissue toxicity after small field hypofractionated stereotactic body radiation. Radiat Oncol 2008;3:36. [Crossref] [PubMed]
- Seung SK, Larson DA, Galvin JM, et al. American College of Radiology (ACR) and American Society for Radiation Oncology (ASTRO) Practice Guideline for the Performance of Stereotactic Radiosurgery (SRS). Am J Clin Oncol 2013;36:310-5. [Crossref] [PubMed]
- Benedict SH, Yenice KM, Followill D, et al. Stereotactic body radiation therapy: the report of AAPM Task Group 101. Med Phys 2010;37:4078-101. [Crossref] [PubMed]
- Li W, Sahgal A, Foote M, et al. Impact of immobilization on intrafraction motion for spine stereotactic body radiotherapy using cone beam computed tomography. Int J Radiat Oncol Biol Phys 2012;84:520-6. [Crossref] [PubMed]
- Ling CC, Humm J, Larson S, et al. Towards multidimensional radiotherapy (MD-CRT): biological imaging and biological conformality. Int J Radiat Oncol Biol Phys 2000;47:551-60. [Crossref] [PubMed]
- Kepenekian V, Muller A, Valette PJ, et al. Evaluation of a strategy using pretherapeutic fiducial marker placement to avoid missing liver metastases. BJS Open 2019;3:344-53. [Crossref] [PubMed]
- Park SH, Won HJ, Kim SY, et al. Efficacy and safety of ultrasound-guided implantation of fiducial markers in the liver for stereotactic body radiation therapy. PLoS ONE 2017;12: [Internet]. [Crossref] [PubMed]
- Oldrini G, Taste-George H, Renard-Oldrini S, et al. Implantation of fiducial markers in the liver for stereotactic body radiation therapy: Feasibility and results. Diagn Interv Imaging 2015;96:589-92. [Crossref] [PubMed]
- Keall PJ, Mageras GS, Balter JM, et al. The management of respiratory motion in radiation oncology report of AAPM Task Group 76. Med Phys 2006;33:3874-900. [Crossref] [PubMed]
- Zhao B, Yang Y, Huq M, et al. SU-E-T-397: Interplay Effect of Gated Lung Stereotactic Body Radiotherapy with RapidArc Delivery. Med Phys 2012;39:3795. [Crossref] [PubMed]
- Gierga DP, Brewer J, Sharp GC, et al. The correlation between internal and external markers for abdominal tumors: implications for respiratory gating. Int J Radiat Oncol Biol Phys 2005;61:1551-8. [Crossref] [PubMed]
- Fayad H, Pan T, Clément JF, et al. Technical note: Correlation of respiratory motion between external patient surface and internal anatomical landmarks. Med Phys 2011;38:3157-64. [Crossref] [PubMed]
- Pettersson N, Oderinde OM, Murphy J, et al. Intrafractional relationship changes between an external breathing signal and fiducial marker positions in pancreatic cancer patients. J Appl Clin Med Phys 2020;21:153-61. [Crossref] [PubMed]
- Vu KN, Haldipur AG, Roh ATH, et al. Comparison of End-Expiration Versus End-Inspiration Breath-Holds With Respect to Respiratory Motion Artifacts on T1-Weighted Abdominal MRI. AJR Am J Roentgenol 2019;1-6. [Crossref] [PubMed]
- Wu WC, Chan CL, Wong YW, et al. A study on the influence of breathing phases in intensity-modulated radiotherapy of lung tumours using four-dimensional CT. Br J Radiol 2010;83:252-6. [Crossref] [PubMed]
- Yang W, Fraass BA, Reznik R, et al. Adequacy of inhale/exhale breathhold CT based ITV margins and image-guided registration for free-breathing pancreas and liver SBRT. Radiat Oncol 2014;9:11. [Crossref] [PubMed]
- Contouring Atlases [Internet]. [cited 2020 Apr 5]. Available online: https://www.rtog.org/CoreLab/ContouringAtlases.aspx
- Lo SS, Fakiris AJ, Chang EL, et al. Stereotactic body radiation therapy: a novel treatment modality. Nat Rev Clin Oncol 2010;7:44-54. [Crossref] [PubMed]
- Kavanagh BD, Timmerman R, Meyer JL. The expanding roles of stereotactic body radiation therapy and oligofractionation: toward a new practice of radiotherapy. Front Radiat Ther Oncol 2011;43:370-81. [Crossref] [PubMed]
- Chance WW, Nguyen QN, Mehran R, et al. Stereotactic ablative radiotherapy for adrenal gland metastases: factors influencing outcomes, patterns of failure, and dosimetric thresholds for toxicity. Pract Radiat Oncol 2017;7:e195-203. [Crossref] [PubMed]
- Ahmed KA, Caudell JJ, El-Haddad G, et al. Radiosensitivity Differences Between Liver Metastases Based on Primary Histology Suggest Implications for Clinical Outcomes After Stereotactic Body Radiation Therapy. Int J Radiat Oncol Biol Phys 2016;95:1399-404. [Crossref] [PubMed]
- Dagoglu N, Callery M, Moser J, et al. Stereotactic Body Radiotherapy (SBRT) Reirradiation for Recurrent Pancreas Cancer. J Cancer 2016;7:283-8. [Crossref] [PubMed]
- Alongi F, Arcangeli S, Filippi AR, et al. Review and uses of stereotactic body radiation therapy for oligometastases. Oncologist 2012;17:1100-7. [Crossref] [PubMed]
- Alongi F, Mazzola R, Figlia V, et al. Stereotactic body radiotherapy for lung oligometastases: Literature review according to PICO criteria. Tumori 2018;104:148-56. [Crossref] [PubMed]
- Rwigema JM, King C, Wang PC, et al. Stereotactic body radiation therapy for abdominal and pelvic oligometastases: Dosimetric targets for safe and effective local control. Pract Radiat Oncol 2015;5:e183-91. [Crossref] [PubMed]
- Alongi F, Fiorentino A, De Bari B. SBRT and extreme hypofractionation: A new era in prostate cancer treatments? Rep Pract Oncol Radiother 2015;20:411-6. [Crossref] [PubMed]
- Milano MT, Katz AW, Okunieff P. Patterns of recurrence after curative-intent radiation for oligometastases confined to one organ. Am J Clin Oncol 2010;33:157-63. [Crossref] [PubMed]
- Milano MT, Philip A, Okunieff P. Analysis of patients with oligometastases undergoing two or more curative-intent stereotactic radiotherapy courses. Int J Radiat Oncol Biol Phys 2009;73:832-7. [Crossref] [PubMed]
- Timmerman RD. An overview of hypofractionation and introduction to this issue of seminars in radiation oncology. Semin Radiat Oncol 2008;18:215-22. [Crossref] [PubMed]
- Grimm J, LaCouture T, Croce R, et al. Dose tolerance limits and dose volume histogram evaluation for stereotactic body radiotherapy. J Appl Clin Med Phys 2011;12:3368. [Crossref] [PubMed]
- sabr uk consortium guidelines [Internet]. [cited 2020 Apr 5]. Available online: https://www.sabr.org.uk/wp-content/uploads/2019/04/SABRconsortium-guidelines-2019-v.6.1.0.pdf
- Miften M, Olch A, Mihailidis D, et al. Tolerance limits and methodologies for IMRT measurement-based verification QA: Recommendations of AAPM Task Group No 218. Med Phys 2018;45:e53-83. [Crossref] [PubMed]
- Zeidan OA, Langen KM, Meeks SL, et al. Evaluation of image-guidance protocols in the treatment of head and neck cancers. Int J Radiat Oncol Biol Phys 2007;67:670-7. [Crossref] [PubMed]
- de Boer HC, Heijmen BJ. A protocol for the reduction of systematic patient setup errors with minimal portal imaging workload. Int J Radiat Oncol Biol Phys 2001;50:1350-65. [Crossref] [PubMed]
- Jaccard M, Champion A, Dubouloz A, et al. Clinical experience with lung-specific electromagnetic transponders for real-time tumor tracking in lung stereotactic body radiotherapy. Phys Imaging Radiat Oncol 2019;12:30-7. [Crossref] [PubMed]
- Bertholet J, Knopf A, Eiben B, et al. Real-time intrafraction motion monitoring in external beam radiotherapy. Phys Med Biol 2019;64:15TR01 [Crossref] [PubMed]
- James J, Cetnar A, Dunlap NE, et al. Technical Note: Validation and implementation of a wireless transponder tracking system for gated stereotactic ablative radiotherapy of the liver. Med Phys 2016;43:2794-801. [Crossref] [PubMed]
- Robin TP, Jones BL, Goodman KA. Fiducial Markers are Necessary for Accurate Delivery of Liver SBRT. Int J Radiat Oncol Biol Phys 2017;99:S222. [Crossref]
- Goldsmith C, Green MM, Middleton B, et al. Evaluation of CyberKnife® Fiducial Tracking Limitations to Assist Targeting Accuracy: A Phantom Study with Fiducial Displacement. Cureus 2018;10:e3523 [Crossref] [PubMed]
- Weksberg DC, Yang JN, Tam AL, et al. Prospective validation of treatment accuracy using implanted fiducial markers for spinal stereotactic body radiation therapy. J Radiosurg SBRT 2016;4:7-14. [PubMed]
- Seppenwoolde Y, Wunderink W, Wunderink-van Veen SR, et al. Treatment precision of image-guided liver SBRT using implanted fiducial markers depends on marker-tumour distance. Phys Med Biol 2011;56:5445-68. [Crossref] [PubMed]
- Wunderink W, Méndez Romero A, Seppenwoolde Y, et al. Potentials and limitations of guiding liver stereotactic body radiation therapy set-up on liver-implanted fiducial markers. Int J Radiat Oncol Biol Phys 2010;77:1573-83. [Crossref] [PubMed]
- Peng JL, Kahler D, Li J, et al. Characterization of a real-time surface image-guided stereotactic positioning system. Med Phys 2010;37:5421-33. [Crossref] [PubMed]
- Jermoumi M, Cao D, Mehta V, et al. SU-G-JeP4-07: Evaluation of Intrafraction Motion Using 3D Surface Guided Radiation Therapy in Lung SBRT. Med Phys 2016;43:3682-3. [Crossref]
- Korreman S, Rasch C, McNair H, et al. The European Society of Therapeutic Radiology and Oncology-European Institute of Radiotherapy (ESTRO-EIR) report on 3D CT-based in-room image guidance systems: a practical and technical review and guide. Radiother Oncol 2010;94:129-44. [Crossref] [PubMed]
- Purdie TG, Bissonnette JP, Franks K, et al. Cone-beam computed tomography for on-line image guidance of lung stereotactic radiotherapy: localization, verification, and intrafraction tumor position. Int J Radiat Oncol Biol Phys 2007;68:243-52. [Crossref] [PubMed]
- Potters L, Kavanagh B, Galvin JM, et al. American Society for Therapeutic Radiology and Oncology (ASTRO) and American College of Radiology (ACR) practice guideline for the performance of stereotactic body radiation therapy. Int J Radiat Oncol Biol Phys 2010;76:326-32. [Crossref] [PubMed]
- Guckenberger M, Meyer J, Wilbert J, et al. Cone-beam CT based image-guidance for extracranial stereotactic radiotherapy of intrapulmonary tumors. Acta Oncol 2006;45:897-906. [Crossref] [PubMed]
- Wang L, Feigenberg S, Fan J, et al. Target repositional accuracy and PTV margin verification using three-dimensional cone-beam computed tomography (CBCT) in stereotactic body radiotherapy (SBRT) of lung cancers. J Appl Clin Med Phys 2012;13:3708. [Crossref] [PubMed]
- Sweeney RA, Seubert B, Stark S, et al. Accuracy and inter-observer variability of 3D versus 4D cone-beam CT based image-guidance in SBRT for lung tumors. Radiat Oncol 2012;7:81. [Crossref] [PubMed]
- Rit S, Nijkamp J, van Herk M, et al. Comparative study of respiratory motion correction techniques in cone-beam computed tomography. Radiother Oncol 2011;100:356-9. [Crossref] [PubMed]
- Haga A, Kida S, Saotome N, et al. Others: Four-dimensional Cone-Beam CT During SBRT. In: Nagata Y. editor. Stereotactic Body Radiation Therapy: Principles and Practices. Tokyo: Springer Japan, 2015:225-36.
- Guckenberger M, Krieger T, Richter A, et al. Potential of image-guidance, gating and real-time tracking to improve accuracy in pulmonary stereotactic body radiotherapy. Radiother Oncol 2009;91:288-95. [Crossref] [PubMed]
- Rock L, Marshall C, McHugh E, et al. Evaluation of the use of average intensity projection for the localisation of mobile lung tumours treated using stereotactic ablative body radiosurgery (SABR). Phys Medica Eur J Med Phys 2014;30:723. [Crossref]
- Vergalasova I, Maurer J, Yin FF. Potential underestimation of the internal target volume (ITV) from free-breathing CBCT. Med Phys 2011;38:4689-99. [Crossref] [PubMed]
- Harris W, Wang C, Yin FF, et al. A Novel method to generate on-board 4D MRI using prior 4D MRI and on-board kV projections from a conventional LINAC for target localization in liver SBRT. Med Phys 2018;45:3238-45. [Crossref] [PubMed]
- Al-Ward S, Wronski M, Ahmad SB, et al. The radiobiological impact of motion tracking of liver, pancreas and kidney SBRT tumors in a MR-linac. Phys Med Biol 2018;63:215022 [Crossref] [PubMed]
- Giaj-Levra N, Niyazi M, Figlia V, et al. Feasibility and preliminary clinical results of linac-based Stereotactic Body Radiotherapy for spinal metastases using a dedicated contouring and planning system. Radiat Oncol 2019;14:184. [Crossref] [PubMed]
- RefleXion debuts PET-guided radiation therapy [Internet]. Physics World 2018 [cited 2020 Jul 10]. Available online: https://physicsworld.com/a/reflexion-debuts-pet-guided-radiation-therapy/